Plenary
Lectures
The
role of morphology and microscopy in coccidian research
in this genomic/proteomic age
David J. P. Ferguson
Nuffield Department of Pathology, Oxford University,
John Radcliffe Hospital, Oxford, OX3 9DU, UK
The history
of the Coccidia is intimately involved with microscopy.
The parasites are below the resolution of the human
eye (with the exception of giant tissue cysts of certain
Sarcocystis species) therefore microscopy is required
for visualisation and identification of the parasite.
The Coccidia belong to the phylum Apicomplexa, a widespread
and successful group of protozoan parasites which
includes many of medical and veterinary importance.
The family Coccidia is characterised by a life cycle
involving faecal/oral transmission with asexual and
sexual development within the alimentary tract of
the definitive host. To survive the rigors of the
external environment the parasites have evolved a
cystic stage (oocyst) that is released with the faeces
and can survive in the external environment. The appearance
and size of these oocysts are characteristic of a
given species and microscopy of faecal samples has
been used for many years to diagnose infections and
to identify the species involved. This single host
species life cycle is typical of those parasites of
the genus Eimeria responsible for coccidiosis in chickens.
However, a sub-group of the Coccidia (including the
genera Toxoplasma, Neospora, Hammondia, Besnotia and
Sarcocystis) has evolved a more elaborate life cycle
involving intermediate hosts where tissue cysts are
formed in the muscle or brain. In these cyst forming
Coccidia, infection can be transmitted to the definitive
host when ingested with the tissue of the intermediate
host. There are obvious advantages for this complex
life cycle for coccidian parasites of carnivores.
The vital role
of microscopy, especially electron microscopy, can
be inferred from the name of the phylum – the
Apicomplexa. This name is derived from the ultrastructural
identification of a characteristic group of organelles
at the apical end of the infectious stages. Through
the 1970s and 80s straightforward electron microscopy
added greatly to knowledge and understanding of the
Coccidia and the developmental processes involved
in their life cycle. Unfortunately many of the papers
were published before the modern electronic age and
therefore are not readily accessible. More recently
there has been a renaissance of both light and electron
microscopy with the development of new molecular techniques.
A major advance was the application of immunocytochemistry
in which antibodies can be used to identify and localise
specific molecules during the parasite life cycle.
Now light and electron microscopy, in conjunction
with modern genomic and proteomic techniques, plays
a vital part in a multidisciplinary approach to improve
our understanding of Coccidian parasites. A few areas
where microscopy and immunocytochemistry have added
to our knowledge of the Coccidia will be illustrated.
Apical complex
The specific group of organelles found in the anterior
of the infectious stages that give rise to the name
Apicomplexa consist of three distinct structures;
the micronemes (small cigar-shaped structures), the
rhoptries (bulbous structures with ducts running to
the anterior) and the dense granules (spherical structures)
that can also be found in other regions of the parasite
(Fig 1a). In the Coccidia, the infectious stages also
possess an additional organelle, the conoid, which
is a truncated cone comprised of microtubules (Fig
1a). The relative and absolute numbers of the various
organelles can vary between species and even between
the infectious stages in a single species. For example
the merozoites of Eimeria tenella have large numbers
of micronemes but few dense granules in contrast to
the tachyzoites Toxoplasma gondii that have numerous
dense granules but few micronemes. Within the Coccidia,
this group of organelles are believed to play a co-ordinated
role in the infection of new host cells. The contents
of the micronemes are released first and are involved
identification and adherence to suitable host cells.
The content of the rhoptries are released during the
invasion process and some proteins are incorporated
into the parasitophorous vacuole. Finally the dense
granules are secreted into and are believed to modify
the parasitophorous vacuole to facilitate parasite
development (reviewed by Carruthers 2002, Mercier
et al 2005). Using molecular techniques, numerous
new proteins are continually being identified (Zhou
et al 2005, Bradley et al 2005) and by using immuno-light
and electron microscopy it has been possible to identify
the location of certain of these molecules to one
or other of the apical organelles. This can assist
in understanding their biological function. By immuno-light
microscopy, molecules can be localised to the apical
region (Fig 1b) and using immuno-electron microscopy
it is possible to identify the exact organelle they
are located in (Fig 1c). It is possible to localise
unknown apical proteins to the micronemes (MIC proteins),
the rhoptries (ROP proteins) or the dense granules
(GRA proteins). It is also possible using double or
triple labelling, to show that different proteins
are located within the same or different organelles.
For example it can be confirmed that GRA2, 4 and 6
are located within the same dense granule (Fig 1d)
and it is also possible to differentiate between organelles
by their protein content with rhoptries labelled with
anti-ROP2 and dense granules by anti-NTPase, a dense
granule protein (Fig 1e). In elegant studies using
T. gondii, which is most amenable to molecular manipulation,
it has been possible to transfect parasites with fluorescent
(GFP) proteins and identify the factors which specifically
targets proteins to each of the various organelles
(Gubbels and Striepen, 2004).
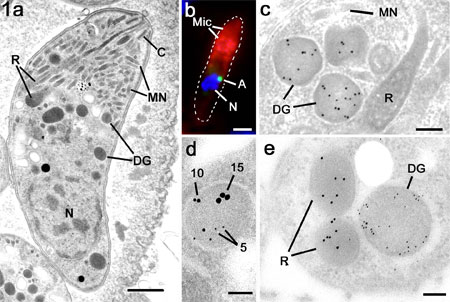
Figure
1a. Electron micrograph of a bradyzoite of
T. gondii showing the characteristic compliment of
apical organelles consisting of the conoid (C), micronemes
(MN), rhoptries (R) and dense granules. N –
nucleus. Bar is 1mm.
b. Immuno-fluorescent image of a
double labelled merozoite of E. tenella showing labelling
of the anterior with anti-MIC2 (Mic; red) and the
small peri-nuclear apicoplast labelled with anti-enoyl
reductase (A; green). Nucleus labelled with DAPI (N;
blue). Bar is 1mm.
c. Immuno-electron micrograph of
a tachyzoite of T. gondii labelled with anti-GRA6
showing the gold particles are limited to the dense
granules (DG) while the micronemes (MN) and rhoptries
(R) and negative. Bar is 100 nm.
d. A triple labelled section using
anti-GRA2 (5 nm gold), anti-GRA4 (10 nm gold) and
anti-GRA6 (15 nm gold) showing a dense granules labelled
with all three markers. Bar is 100 nm.
e. Cross section through the anterior
of a merozoite of T. gondii double labelled with anti-ROP2
(10 nm gold) and anti- NTPase (5 nm gold) showing
the specific labelling of the rhoptries (R) and dense
granules (DG). Bar is 100nm
(Copyright for all images is retained by D J P Ferguson,
Oxford University)
Apicoplast
More recently, the apicomplexan
parasites have also been found to possess a second
unique feature. They contain a non-photosynthetic
plastid, which has been termed the apicoplast (reviewed
by Wilson 2002, Waller and McFadden 2005). This residual
plastid is believed to be derived from an ancient
secondary endosymbiotic acquisition from a red alga
(Wilson 2002). The plastid genome was first identified
in Plasmodium sp. but has subsequently been shown
to be present in all members of the Apicomplexa with
the exception of Cryptosporidium sp. (Xu et al 2004).
It has been shown that the apicoplast is essential
for parasite survival and parasites without an apicoplast
are non-viable. In the absence of a plastid, parasites
displayed an unusual delayed death phenotype (He et
al 2001). That is, the parasites without a plastid
could continue to divide within the host cell vacuole
as long as one plastid containing organism was present.
Indeed they were able to escape and invade new host
cells but were unable to undergo proliferation within
the new host cell resulting in death of the parasite
(He et al, 2001). These findings are extremely exciting
since the prokaryotic nature of this organelle could
represent new and unique targets for drug intervention.
The role of the apicoplast is still incompletely understood
but it is known to be involved in type II fatty acid
biosynthesis and isoprenoid biosynthesis (reviewed
Wilson 2002, Waller and McFadden 2005). By electron
microscopy it was shown that this genome was located
within an organelle characterised by being limited
by multiple membranes (McFadden et al 1996, Kohler
et al 1997) (Fig 2c). This organelle was first identified
by electron microscopy in the 1960s (Sheffield and
Melton 1968) although its function remained unknown.
The exact number of membranes is still controversial
(Kohler 2005) but the presence of multiple (3/4) membranes
allows identification of the organelle and differentiates
it from the other genome containing organelles (the
nucleus and the mitochondrion) that are limited by
two membranes (Fig 2c, e). It has been found that
many of the plastid specific proteins are encoded
by nuclear genes (lateral gene transfer) and these
proteins have to be targeted back to the apicoplast
using a specific bi-partite targeting sequence. Initial
morphological studies of the apicoplast examined,
in vitro, asexual development of parasites transfected
with the green fluorescent protein targeted to the
apicoplast (Striepen et al 2000, Vaishnava et al 2005).
More recently, in vivo studies using antibodies to
an apicoplast specific protein (enoyl reductase) have
examined the changes in the apicoplast during both
asexual and sexual development in T. gondii (Ferguson
et al 2005) and E. tenella. In the infectious
(sporozoite, merozoite, tachyzoite and bradyzoite)
stages the apicoplast is normally present as a single
small spherical structure adjacent to the nucleus
(Fig 1b, 2a) although multiple plastids have been
observed in the merozoites of E. tenella
(Fig 2b). Asexual proliferation (schizogony) results
in the simultaneous formation of numerous daughters.
To ensure their viability, each merozoite must receive
a nucleus and at least one apicoplast and mitochondrion.
This requires a tightly co-ordinated process of nuclear,
apicoplast and mitochondrial division and segregation.
The possible mechanisms are still under investigation.
There are conflicting observations with certain studies
showing a close relationship between nuclear and apicoplast
division (Striepen et al 2000, Vaishnava et al 2005)
while others show evidence that nuclear and apicoplast
division are independent events (Ferguson et al 2005)
(Fig 2d). However, organelle segregation to the daughters
may involve the nuclear pole/centriolar complex in
all cases. When sexual development was examined, there
was found to be little change in the apicoplast during
microgametogony and the microgametes lacked an apicoplast.
In contrast there is a marked increase in the size
and activity of the apicoplast during macrogametogony
perhaps reflecting the increased metabolic activity
(Fig 2f). This means that there will be maternal inheritance
of the apicoplast with the sporozoites, formed within
the oocyst, receiving their apicoplast from the macrogamete
(Ferguson et al 2005).

Figure
2 a and b. Tachyzoite of
T. gondii (a) and merozoite of E. tenella (b) labelled
with anti-enoyl reductase showing the peri-nuclear
location of the single or multiple apicoplasts (arrows;
green). Bar is 1mm.
c. High power electron micrograph
of an apicoplast in E. tenella showing the four limiting
membranes (arrowheads). Bar is 100 nm.
d. Immuno-fluorescent image of multinucleate
schizont of T. gondii in the cat intestine showing
a large elongated and branched apicoplast (arrows).
Bar is 1mm.
e. Electron micrograph of part of
a schizont similar to that in d showing the enlarged
multimembraned apicoplast (A) and the nucleus (N)
and mitochondrion Mi) limited by two membranes. Bar
is 100 nm
f. Immuno-fluorescent image of macrogamete
of T. gondii in the cat intestine showing a large
elongated and branched apicoplast (arrows). Bar is
1mm.
(Copyright for all images is retained by D J P Ferguson,
Oxford University)
Asexual development
Asexual development within
the Coccidia is often considered as a single process,
which has been termed schizogony, only varying in
the number of daughters formed. However when the process
was examined in different species by electron microscopy,
subtle differences could be identified showing at
least four variations in the process exhibited by
different parasites. There is classical schizogony
which is undergone by the vast majority of coccidian
species including the genera Eimeria. It can
be summarised as involving a proliferative phase in
which there are repeated cycles of DNA replication
and nuclear division giving rise to a multinucleate
stage followed by a differentiation phase where daughter
formation is initiated at the parasite surface and
the nuclei and other organelles segregate to the daughters
as they bud into the parasitophorous vacuole (Fig
3). A variation of this process is undergone by the
coccidian stages of T. gondii in the cat gut where
there is an identical proliferative phase but during
the differentiation phase daughter formation is initiated
and completed within the mother cell cytoplasm (Ferguson
et al 1974, 2005) and this process has been termed
endopolygeny (Fig 3). A third variation is that undergone
by certain asexual stages of Sarcocystis spp. where
there are repeated cycles of DNA replication but with
no nuclear division. In this case the initiation of
multiple daughter formation occurs within the mother
cell cytoplasm and coincides with fragmentation of
the polyploid nucleus into a number of haploid nuclei
each of which enters a developing daughter in a similar
manner to that seen in endopolygeny (Speer and Dubey
1999, 2001, Vaishnava et al 2005). The fourth variation
is the one that has been most extensively studied
since it is that undergone by the exo-enteric (tachyzoites
and bradyzoites) forms of T. gondii and has been termed
endodyogeny. In this case only two daughters are formed
with the initiation of daughter formation within the
mother cell cytoplasm occurring before completion
of nuclear division (Fig 3). The process of daughter
formation is similar to that observed during endopolygeny
but only two daughters are formed (Sheffield and Melton1968,
Striepen et al 2000). These three variations from
classical schizogony are the exception and it may
be significant that they are found within the sub-group
of cyst forming Coccidia, which have had to adapt
to development in intermediate hosts. Although these
subtle variations can only be identified by electron
microscopy, an appreciation of these differences can
help to reconcile certain apparent conflicting results
in relation to the division and segregation of the
nuclei and apicoplasts during daughter formation in
T. gondii, Sarcocystis sp., and E. tenella
(Striepen et al 2000, Vaishnava et al 2005, Ferguson
et al 2005).
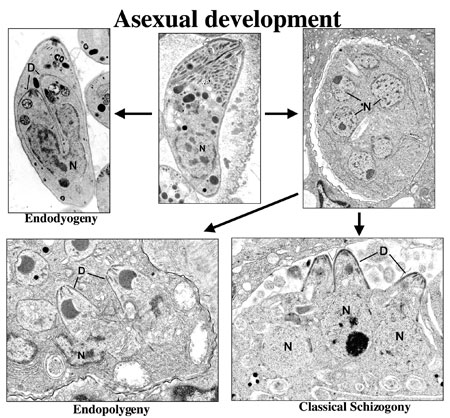
Figure 3a.
A montage of asexual development illustrating certain
of the ultrastructural differences associate with
the process of endodyogeny, endopolygeny and classical
schizogony within the Coccidia.
(Copyright for all images is retained by D J P Ferguson,
Oxford University)
Sexual development
Microgamete formation
Within the Coccidia, microgametogony
results in the formation of relatively low numbers
of microgametes. The relative number of microgametocytes
varies between species. In the case of T. gondii,
where there are relatively few microgametocytes to
the number of macrogametes, it has been questioned
if there are sufficient microgametes to ensure that
efficient fertilisation can take place (Ferguson 2003).
By electron microscopy it is interesting to note just
how similar microgametes are to mammalian sperm. They
consist of a compact, electron dense nucleus, a mitochondrion
and are powered by two large flagella (Fig 4a, b).
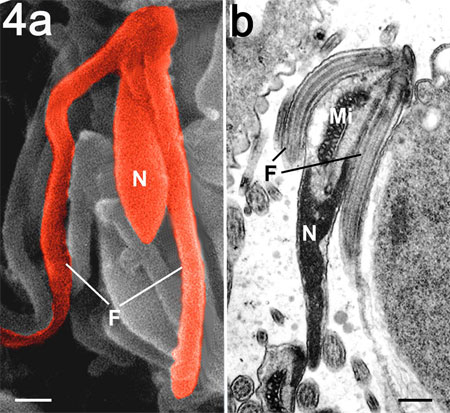
Figure 4.
Scanning (a) and transmission electron
microscopy (b) of the mature microgamete
of T. gondii showing the nucleus (N), mitochondrion
(Mi) and two flagella (F). Bar is 100 nm.
(Copyright for all images is retained by D J P Ferguson,
Oxford University)
Macrogamete and oocyst formation
The survival of this group
of parasites depends on the ability of the oocysts
formed from the macrogametes to survive for extended
periods in the external environment. Not only that,
but the oocyst have to undergo sporulation to form
eight infectious sporozoites, which is the only example
of extra-cellular development by coccidian parasites.
This places two essential requirements on the developing
macrogamete. The first is that it must acquire and
store all the ingredients necessary to allow sporulation
while cut off from additional nutrients in the hostile
external environment. Thus the macrogamete contains
large amounts of storage material in the form of polysaccharide
granules and lipid droplets (Fig 5a). The second is
for the macrogametocyte to synthesise and store the
material required to form the oocyst wall. The oocyst
wall is a complex structure consisting of a loose
outer veil, which is lost during excretion, and a
wall consisting of two distinct layers, a thick electron
dense outer layer and a thinner more electron lucent
inner layer (Fig 5g). The structure is pretty consistent
across the Coccidia (Ferguson et al, 1975, 1977, 2003).
The report of a thin (10 nm thick) outer layer and
a thicker (90nm) inner layer observed for oocysts
of E. tenella isolated from faeces (Stotish
et al 1978) may represent an example of where part
of the wall has been lost during processing. Any disruption
in the process of oocyst wall formation would have
serious implications for parasite transmission. To
understand this process, genes for certain components
of the oocyst wall have been identified (Belli et
al 2002a, b, 2003, Wallach, 2002). Using antibodies
to these and to other proteins it has been possible
to start to dissect the changes occurring during macrogamete
maturation and oocyst wall formation by combining
electron microscopy and immunocytochemistry (Mouafo
et al. 2002, Ferguson et al, 2003). An accidental
and unexpected finding was that an antibody to the
specific apple domains of the MIC4 protein of T. gondii
(TgMIC4, Brecht et al 2001) cross reacted with a subset
of granules in the early macrogametocyte of T. gondii
(Ferguson et al, 2000) but also with small granules
in the early macrogametocytes of E. maxima
(Ferguson et al, 2003) and E. tenella.
In all three cases, the contents of these granules
were secreted into the parasitophorous vacuole and
appeared to associate with the formation of the loose
outer veil (Fig 6d). These previously unidentified
granules have been termed the veil forming bodies
(VFB) (Ferguson et al 2003). It is interesting to
speculate that the veil contains a protein with apple
domains and, since these domains are associated with
protein-protein or protein-carbohydrate interactions,
they could be involved in microgamete recognition.
The second type of granule is the large electron dense
membrane bound spherical structures, which have been
termed the wall forming bodies type 1 (WFB1) (Fig
5a, g). In addition, there is a third structure consisting
of irregular shaped electron dense deposits located
with the rough endoplasmic reticulum that have been
identified as the wall forming bodies type 2 (WFB2)
(Fig 5a, g). Using immuno-light and electron microscopy
is was possible to identify and follow the changes
in these structures during oocyst wall formation.
Antibodies to affinity purified gametocyte antigens
(anti-APGA, Belli et al 2002a, b) stained the WFB1
strongly and the WFB2 weakly (Fig 5c) and it was possible
to follow the secretion of the WFB1 to form the outer
layer of the oocyst wall (Fig 5e, f). In contrast,
the anti-gam56 (Belli et al 2002b) and anti-gam82
(Belli et al, 2003a) antibodies only stained WFB2
(Fig 5d), the contents of which were released to form
the inner layer of the oocyst wall (Fig 5f). The question
of how material located in the endoplasmic reticulum
could be secreted remained. However, by immuno-EM,
it was observed that, only after the release of the
contents of WFB1, the WFB2 material retained in the
rER was transferred to the surface via the Golgi body
(Ferguson et al, 2003). This sophisticated control
mechanism allows independent and sequential secretion
of the three components thus allowing efficient formation
of the stratified oocyst wall. These results were
obtained using antibodies to Eimeria maxima
proteins but there is likely to be close homology
between the genes within the genus Eimeria
and indeed it was found that these antibodies cross
reacted with the homologous proteins of E.
tenella and gave a similar staining pattern.
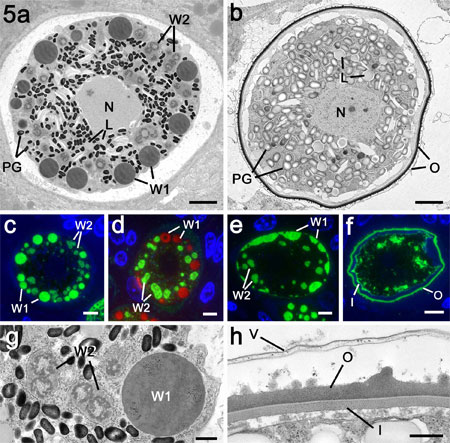
Figure 5a.
Electron micrograph through a mature macrogamete of
E. maxima showing the central nucleus and the cytoplasm
packed with numerous polysaccharide granules (PG)
and lipid droplet (L) in addition to the WFB1 (W1)
and the WFB2 (W2). Bar is 1mm.
b. Sections through an early oocyst
with a fully formed oocyst wall. Note that while the
polysaccharide granules (PG) and lipid droplets (L)
are still present but both types of WFB had disappeared.
Bar is 1mm.
c. Immuno-fluorescent images through
a mature macrogamete stained with anti-APGA showing
strong labelling of the WFB1 (W1) and lighter staining
of the WFB2 (W2). Bar is 1mm.
d. Similar organism to that in c
stained with anti-gam56 showing that only the WBF2
(W2) are stained. The WFB1 (W1) were visualised by
Evans blue staining (red). Bar is 1mm.
e. Early stage of oocyst wall formation
showing secretion of the WFB1 to initiate formation
of the outer layer of the oocyst wall (W1). Stained
with anti-APGA. W2 – WFB2. Bar is 1mm.
f. Late stage in oocyst wall formation
in which both the inner (I) and outer (O) layers are
formed. Stained with anti-APGA. Bar is 1mm.
g. Detail from the macrogamete in
a showing the large membrane bound WFB1 (W1) and the
smaller irregular clumps of material representing
the WBF2 (W2) located within the rER. Bar is 0.5 mm.
h. Detail of the fully formed oocyst
wall consisting of the loosely attached outer veil
(V) along with the electron dense outer layer (O)
and the thinner and less electron dense inner layer
(I). Bar is 0.5 mm.
(Copyright for all images is retained by D J P Ferguson,
Oxford University)
The process of fertilisation
is yet to be observed in detail but it would appear
that oocyst wall formation occurs prior to release
from the host cell (Fig 6b). An extensive search failed
to show microgametes associated with the macrogametes
even when located in adjacent cells (Fig 6a). However,
a high proportion of oocysts in the lumen were found
to have attached microgametes (Fig 6c). These were
located beneath the veil adjacent to the outer layer
of the wall (Fig 6d). It is still not clear when and
how the microgamete completes fertilisation.
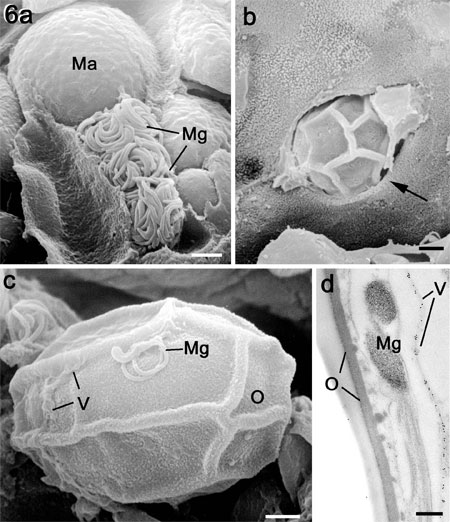
Figure
6a. Scanning electron micrograph (SEM) of
a fracture through a caecum infected with E. tenella
showing a microgametocyte with numerous mature microgametes
(Mg) in a cell adjacent to a macrogamete (Ma). Bar
is1 mm.
b. SEM of the luminal surface of
a crypt showing an oocyst (characterised by the surface
folds) escaping from and epithelial cell (arrow).
Bar is1 mm.
c. SEM of an oocyst in the gut lumen
showing partial loss of the veil (V) and with a microgamete
(Mg) adhering to the outer layer of the oocyst wall
(O). Bar is1 mm.
d. Transmission electron micrograph
through the surface of an oocyst showing the microgamete
(Mg) located between the veil (labelled with anti-TgMIC5)
(V) and the outer layer (O) of the oocyst wall. Bar
is 100nm.
(Copyright for all images is retained by D J P Ferguson,
Oxford University)
This summarises a few examples where microscopy, combined
with immunocytochemistry, has helped in our understanding
of the Coccidia. There are still many areas where
further research, using molecular techniques and microscopy,
will provide new insights and perhaps identify areas
of weakness in this important group of parasites.
Acknowledgements
The work described in this
paper has only been possible because of the active
collaboration of a large number of people from the
research groups of Rima McLeod (Chicago University,
USA), Craig Roberts (Strathclyde University, UK),
John Boothroyd (Stanford University), USA, Fiona Tomley
and Martin Shirley (Compton, UK), Sabina Belli and
Nick Smith (University of Technology, Sydney), Dominique
Soldati (Geneva, Switzerland), J-F Dubremetz (Montpellier,
France) and M-F Cesbron-Delauw (La Tronche, France).
DJPF was supported by an equipment grant from the
Wellcome Trust. The work on the structure of the macrogamete
and oocyst was supported by ABIC Ltd.
References
Belli SI, Lee M, Thebo
P, Wallach MG, Schwartsburd B, Smith NC, 2002a. Biochemical
characterisation of the 56 and 82 kDa immunodominant
gametocyte antigens from Eimeria maxima. Int J Parasitol.
32, 805-16.
Belli SI, Wallach MG, Smith NC. 2003. Cloning and
characterization of the 82 kDa tyrosine-rich sexual
stage glycoprotein, GAM82, and its role in oocyst
wall formation in the apicomplexan parasite, Eimeria
maxima. Gene 307, 201-12.
Belli SI, Witcombe
D, Wallach MG, Smith NC, 2002b. Functional genomics
of gam56: characterisation of the role of a 56 kilodalton
sexual stage antigen in oocyst wall formation in Eimeria
maxima. Int J Parasitol.32,1727-37.
Bradley PJ, Ward C, Cheng SJ, Alexander DL, Coller
S, Coombs GH, Dunn JD, Ferguson DJP, Sanderson SJ,
Wastling JM, Boothroyd JC. Proteomic analysis of rhoptry
organelles reveals many novel constituents for host-parasite
interactions in Toxoplasma gondii. J Biol. Chem. Jul
7; [Epub ahead of print]
Brecht S, Carruthers VB, Ferguson DJP, Giddings OK,
Wang G, Jackle U, Sibley LD, Soldati D. 2001. The
Toxoplasma microneme protein MIC4 is an adhesin composed
of six conserved apple domains J. Biol. Chem. 276,
4119-27.
Carruthers VB. 2002. Host cell invasion by the opportunistic
pathogen Toxoplasma gondii. Acta Trop. 81:111-22.
Ferguson DJP. 2002 Toxoplasma gondii and sex: essential
or optional extra. Trends in Parasitology 18: 355-359.
Ferguson DJP, Belli SI, Smith NC, Wallach MG. 2003.
The development of the macrogamete and oocyst wall
in Eimeria maxima: Immuno-light and electron microscopy.
Inter J Parasitol 33: 1329-1340, 2003.
Ferguson DJP, Birch-Andersen A, Hutchison WM, Siim
JChr. 1977. Ultrastructural studies on the endogenous
development of Eimeria brunetti IV. Formation and
structure of the oocyst wall. Acta Path Microbiol
Scand, Sect B 85: 201-211.
Ferguson DJP, Brecht S, Soldati D. 2000. The microneme
protein MIC4, or a MIC4-like protein, is expressed
within the macrogamete and associated with oocyst
wall formation in Toxoplasma gondii. Int. J Parasitol.
30,203-09.
Ferguson DJP, Hutchison WM, Dunachie JF and Siim J
Chr. 1974. Ultrastructural study of early stages of
asexual multiplication and microgametogony of Toxoplasma
gondii in the small intestine of the cat. Acta Path
Microbiol Scand, Sect B 82: 167-181.
Ferguson DJP, Hutchison WM, Siim, JChr. 1975. The
ultrastructural development of the macrogamete and
formation of the oocyst wall of Toxoplasma gondii.
Acta Path. Microbiol. Scand., Sect. B 83, 491-505.
Gubbels MJ, Striepen B. 2004. Studying the cell biology
of apicomplexan parasites using fluorescent proteins.
Microsc Microanal.10:568-79.
He, CY, Shaw MK, Pletcher CH, Striepen B, Tilney LG,
Roos DS. 2001. A plastid segregation defect in the
protozoan parasite Toxoplasma gondii. EMBO J. 20:330-9.
Kohler S. 2005. Multi-membrane-bound structures of
Apicomplexa: I. the architecture of the Toxoplasma
gondii apicoplast. Parasitol Res. 96:258-72.
Kohler S, Delwiche CF, Denny PW, Tilney LG, Webster
P, Wilson PRG, Palmer JD, Roos DS. 1997. A plastid
of probable green algal origin in Apicomplexan parasites.
Science 275:1485-9.
McFadden, GI, Reith ME, Munholland J, Lang-Unnasch
N. 1996. Plastid in human parasites. Nature 381:482.
Mercier C, Adjogble KD, Daubener W, Delauw MF. 2005.
Dense granules: Are they key organelles to help understand
the parasitophorous vacuole of all apicomplexa parasites?
Int J Parasitol. 35:829-49.
Mouafo AN, Weck-Heimann A, Dubremetz J-F, Entzeroth
R. 2002. Monoclonal antibodies specific for the two
types of wall-forming bodies of Eimeria tenella macrogametes
(Coccidia, Apicomplexa). Parasitol. Res. 88, 217-24.
Sheffield, HG., Melton ML. 1968. The fine structure
and reproduction of Toxoplasma gondii. J. Parasitol.
54: 209-226.
Speer CA, Dubey JP. 1999. Ultrastructure of shizonts
and merozoites of Sarcocystis falcatula in the lungs
of budgerigars (Melopsittacus undulatus). J Parasitol.
85:630-7.
Speer CA, Dubey JP. 2001. Ultrastructure of schizonts
and merozoites of Sarcocystis neurona. Vet Parasitol.
95:263-71.
Stotish RL, Wang CC, Meyenhofer M. 1978. Structure
and composition of the oocyst wall of Eimeria tenella.
J Parasitol. 64:1074-81.
Striepen B, Crawford MJ, Shaw MK, Tilney LG, Seeber
F, Roos DS. 2000. The plastid of Toxoplasma gondii
is divided by association with the centrosomes. J.
Cell Biol. 151:1423-34.
Vaishnava1 S, Morrison DP, Rajshekhar Y. Gaj RY, Murray
JM, Entzeroth R, Howe DK, Striepen B. 2005. Plastid
segregation and cell division in the apicomplexan
parasite Sarcocystis neurona. J Cell Sci. (in press)
Wallach, M. 2002. The development of CoxAbic®
a novel vaccine against coccidiosis. World Poultry
18, 2-4
Waller RF, McFadden GI.2005. The apicoplast: a review
of the derived plastid of apicomplexan parasites.
Curr Issues Mol Biol. 7:57-79.
Wilson, RJM. 2002. Progress with parasite plastids.
J. Mol. Biol. 319:257-274.
Xu P, Widmer G, Wang Y, Ozaki LS, Alves JM, Serrano
MG, Puiu D, Manque P, Akiyoshi D, Mackey AJ, Pearson
WR, Dear PH, Bankier AT, Peterson DL, Abrahamsen MS,
Kapur V, Tzipori S, Buck GA. 2004. The genome of Cryptosporidium
hominis. Nature. 431:1107-12.
Zhou XW, Kafsack BF, Cole RN, Beckett P, Shen RF,
Carruthers VB. 2005. The opportunistic pathogen Toxoplasma
gondii deploys a diverse legion of invasion and survival
proteins. J Biol Chem. 2005 Jul 7; [Epub ahead of
print].