Plenary
Lectures
NEW ROLES FOR
PROTEASES IN THE INFECTION BIOLOGY OF COCCIDIAN PARASITES
Fabiola Parussini1, Chin
Fen Teo1, Jill M. Harper1, Emily Binder2, Kami Kim2,
Vern B. Carruthers1
1W. Harry Feinstone Department of Molecular Microbiology
and Immunology, Johns Hopkins Bloomberg School of
Public Health, 615 N. Wolfe Street, Baltimore, MD
21205 USA
e-mail: vcarruth@jhsph.edu
2 Departments of Medicine and of Microbiology and
Immunology, Albert Einstein College of Medicine, 1300
Morris Park Ave, Bronx, NY 10461 USA
Summary
Coccidian parasites including
Toxoplasma gondii cause widespread infections and
disease in humans and animals. The need for new therapeutic
agents along with the fascinating biology of these
parasites has fueled a keen interest in understanding
how key steps in the life cycle are regulated. Proteolysis
is intimately associated with cell and tissue invasion
by these obligate intracellular parasites and recent
studies have begun to identify the proteases involved
in these processes. Based on clues from inhibitor
experiments and cleave site mapping studies, we and
others are using emerging genome information and molecular
genetics to identify and validate proteases that regulate
secretory organelle biogenesis and invasion protein
activity. These studies are revealing roles for a
variety of proteases including cathepsins, subtilases,
and rhomboids in cell and tissue invasion. The identification
of highly selective inhibitors for these proteases
has the potential to not only further dissect their
role in infection but also to potentially prevent
or ameliorate disease.
Introduction
Coccidian parasites are
responsible for several important animal and human
diseases. Neospora caninum is a leading cause of spontaneous
abortion in bovines, leading to significant economic
losses in the dairy industry (1). Eimeria infection
of commercial livestock remains an important source
of production losses (2), particularly in the poultry
industry where high density facilities provide an
ideal environment for rampant spread of disease. Toxoplasma
gondii commonly infects animals and humans, causing
birth defects, ocular disease, and neurological complications,
particularly in immunocompromised individuals (3).
Collectively these parasitic infections have multi-billion
dollar yearly economic impact due to production losses
and the medical care and institutionalization of afflicted
people.
Although a variety of antibiotics are currently used
to treat coccidian infections, these anti-coccidial
compounds remain hampered by limitations. Eimeria
drug resistance or tolerance is an ongoing problem
in poultry production facilities. Although drug resistance
in Toxoplasma infections is uncommon, front line treatments
such as antifolate combination therapy (e.g., pyrimethamine
and sulfadiazine) are often poorly tolerated or cause
severe allergic reactions in patients. These limitations,
combined with the fascinating properties of coccidian
parasites, have accelerated interest in investigating
mechanisms governing the infection biology of these
pathogens.
Most of the attention has focused on unique aspects
of coccidian parasites that are distinct from their
host or other eukaryotes because these exceptional
features and properties represent both potential targets
for therapeutic intervention and opportunities to
expand the boundaries of biological insight. Cell
invasion is a unique and essential property of coccidian
parasites that has received particularly intense attention
because of its dramatic features. Typically complete
within 10-20 seconds, coccidian zoites power their
way into susceptible host cells by using a unique
form of locomotion, gliding motility. Molecular interrogations
of gliding motility and host cell invasion have recently
revealed that proteases play central roles several
distinct aspects of these processes. This article
is intended to highlight new and emerging insight
into the function of motility and invasion proteases
in coccidian parasites.
Invasion
organelles and proteins
Because of its experimental
tractability, Toxoplasma has been most extensively
investigated coccidian parasite for invasion studies.
Like other coccidians, Toxoplasma actively invades
host cells in a process that is largely parasite driven
and distinct from the facilitated endocytosis mechanisms
used by many intracellular bacteria and viruses. The
parasite uses gliding motility to approach a target
host cell and slide along its surface. Gliding motility
is driven by the parasite’s intrapellicular
actin-myosin motor system (4), which connects both
with the inner membrane complex (5) and transmembrane
adhesive proteins secreted from micronemes (6). Micronemes
are small cigar-shaped secretory organelles that discharge
their contents by fusion at the extreme apical end
of the parasite (7). Basal secretion of micronemes
occurs continuously as the parasite glides over surfaces
by treadmilling microneme adhesive proteins from the
apical tip to posterior pole. Upon reaching a suitable
site for invasion, the parasite further relies of
sequential discharge of secretory organelles to mediate
entry (8). In the first event, microneme contents
accumulate on the parasite apical surface where they
bind host receptors during a step that coincides with
the formation of the moving junction, a tight apposition
of the parasite and host plasma membranes. Immediately
thereafter, the contents of the club-shaped rhoptries
are extruded through an apical duct where they translocate
into the host cell. Underneath the site of invasion,
rhoptry proteins and lipids reconfigure into small
vesicles (evacuoles) that fuse with the parasitophorous
vacuole (PV) as the parasite plunges into the cell
(9). Similar to gliding motility, entry is powered
by the actin-myosin depend translocation of microneme
adhesins backward across the parasite surface to the
posterior end where they accumulate in a “cap-like”
structure before being shed during the last few seconds
of penetration (10). Although the PV is created by
pushing in the host plasma membrane, the moving junction
excludes most of the host surface components and this
is thought to be a key survival strategy for avoiding
acidification and fusion with the endocytic or lysosomal
systems. Within a few minutes of entry, a third class
of secretory organelles called dense granules (DG)
are released by fusion with the apical membrane. DG
proteins extensively modify the PV and are thought
to participate in nutrient acquisition. Although DGs
resemble regulated dense core secretory granules of
higher eukaryotes, their secretion is not as tightly
controlled as micronemes and rhoptries. This, along
with evidence that DGs are a “default”
pathway through which proteins traffic in the absence
of specific forward targeting signals (11), suggests
that DGs are not classic regulated secretory organelles.
Interestingly, among the three classes of secretory
proteins associated with invasion, DG proteins are
the only group that is not proteolytically processed.
Thus, proteolysis is tightly associated with micronemes
and rhoptries, the two pathways that are most intimately
coupled with parasite invasion.
Understanding
the role of proteases in cell and tissue invasion:
from proteolytic events and inhibitors to candidate
enzymes
Two main tacks have been
used to uncover evidence of proteases involvement
in coccidian invasion. One approach has been to identify
proteolytically processed invasion proteins and map
their cleavage sites. Although usually not definitive,
cleavage site information can often provide clues
about the type of protease involved based on the known
recognition specificities of different classes of
proteases. A second approach is the use of class specific
protease inhibitors. For example, the serine protease
inhibitors 3,4 dichloroisocumarin (3,4 DCI) and 4-(2-Aminoethyl)
benzenesulphonyl fluoride (AEBSF) have been reported
to block Toxoplasma invasion of human fibroblast cells
(12). Other serine protease inhibitors similarly block
Eimeria invasion (13,14). Cysteine proteases
have also been implicated in invasion based on findings
that cysteine protease inhibitor (PRT2253F) with activity
against cathepsin B inhibits Toxoplasma cell entry
(15). Although, limited by the possibility of non-specific
“off target” effects, these studies at
least provided initial evidence for the role of proteolysis
in coccidian invasion. The identification and analysis
of proteolytic substrates and the use of protease
inhibitors have revealed that proteases function in
several distinct steps of invasion (Fig. 1). This
section will discuss how these approaches, along with
the recent completion of the Toxoplasma genome, have
recently led to the identification of candidate enzymes
involved in each step.
Figure 1.
Sites of invasion protein proteolysis
Microneme and rhoptry invasion proteins are subjected
to a series of proteolytic processing steps including:
(1) Proteolytic maturation en route to secretory organelles;
(2) Mobilization to the parasite surface during parasite
attachment; (3) Primary processing that trims microneme
proteins while they are expressed on the parasite
surface; and (4) Shedding by which the microneme products
are eventually released from the parasite surface
after they translocate toward the posterior end. Protease
inhibitors that block each of these steps are listed.
Proteolytic
maturation of invasion proteins
While performing pulse-chase
metabolic labeling experiments, Achbarou (16) and
Soldati (17) noted that nascent microneme (TgMIC3)
and rhoptry (TgROP1) proteins are proteolytically
processed within minutes of their initial translation.
It is now appreciated that proteolytic maturation
is a widespread phenomenon associated with most invasion
proteins destined for secretion via the micronemes
or rhoptries (Fig. 2). Such proteins are initially
synthesized as preproproteins. The “pre”
segment is the signal peptide, which is removed cotranslationally
by signal peptidase during import into the endoplasmic
reticulum. The “pro” peptide is subsequently
removed as the protein transits through the secretory
pathway. Rhoptry proteins appear to undergo processing
in the nascent rhoptries during parasite cell division
by endodyogeny (17,18). To investigate the sub-cellular
site of microneme processing, we generated antibodies
to the propeptides of TgMIC5 and TgM2AP. Immunofluorescent
staining of extracellular or intracellular parasites
revealed that these precursors occupy the trans-Golgi
network and an early endosome compartment defined
by co-staining with Rab51. No staining of mature micronemes
was seen, however, some parasites showed partial localization
of TgMIC5 within the DGs suggesting this may be an
alternative route for secretion of immature microneme
proteins. Collectively, our findings imply that microneme
protein maturation occurs within or just beyond the
early endosome, and not within in mature micronemes.
What roles do propeptides play in the biogenesis of
secretory organelles? Although this remains an incompletely
resolved question, recent evidence suggests that propeptides
assist in the trafficking and regulation of their
cognate proteins. For example, the TgROP1 propeptide
and a segment containing the TgROP4 propeptide both
supported the trafficking of heterologous proteins
to the rhoptries (19,20). It should be noted, however,
that TgROP1 can also use alternative sorting signals
since a propeptide deletion mutant was still correctly
targeted to the rhoptries (17). Also, based on analysis
of a non-cleavable site directed mutant, processing
of TgROP1 is not necessary for its trafficking or
for rhoptry biogenesis (21). However, since ROP1 is
not an essential protein (22) and only one of many
that are trafficked to the rhoptries, it may not be
ideal for assessing a general role of propeptides
in organellar biogenesis. On the other hand, protease
inhibitors are capable of interfering with the processing
of multiple substrates cleaved by a single protease
and therefore are expected to have more profound effects.
Consistent with this notion, Shaw et al. (23) showed
that subtilisin inhibitor III and cathepsin inhibitor
III block parasite replication and cause marked abnormalities
of secretory compartments including the rhoptries.
Based in part on this study, Miller and coworkers
(24) investigated a role for TgSUB2 in propeptide
processing. TgSUB2 is a member of the subtilase family
of serine proteases. Although Toxoplasma harbors at
least 12 subtilase genes, most of these have not been
investigated in any detail. TgSUB2 undergoes autocatalytic
processing en route to the rhoptries and mutation
of the natural autocleavage site revealed a key role
for and acidic residue at the P1 position (cleavage
site residues are designated P4-P3-P2-P1 / P1’-P2’-P3’-P4’,
where / indicates the scissile site). Interestingly,
an acidic P1 residue is also required for ROP1 processing
and TgSUB2 co-immunoprecipitates with ROP1. Also,
ROP2, 4, and 8 have similar putative cleavage sites,
suggesting they are candidate substrates for TgSUB2.
However, a cysteine protease of the cathepsin family,
TgCPB (also called TgCP1 or Toxopain 1), has also
been implicated in rhoptry protein processing based
on its trafficking to the rhoptries and on the observations
that a cathepsin B inhibitor partially blocks ROP2,3,4
processing, disrupts rhoptry biogenesis, and interferes
with parasite invasion and infection (15,25). Thus,
it appears that Toxoplasma expresses at least two
distinct rhoptry maturases that participate in the
processing of rhoptry proteins and in rhoptry biogenesis.
Propeptides appear to also play a central role in
the trafficking and regulation of microneme proteins.
TgMIC3 uses a short N-terminal propeptide to mask
the carbohydrate binding activity of its lectin-like
domain (26). This presumably prevents inappropriate
binding to parasite glycoproteins within the secretory
pathway. A propeptide deletion mutant (Dpro) of TgMIC3
also fails to reach the micronemes and is instead
retained within the secretory pathway along with its
partner protein TgMIC8 (M. Lebrun, personal communication).
Similarly, we have shown that DproTgMIC5 and DproTgM2AP
are retained within or near the early endosome, in
addition to other sites. These findings indicate that
microneme propeptides function in the trafficking
of their cognate proteins, possibly by binding to
cargo receptors. Interestingly, TgM2AP’s partner
protein TgMIC2 also contains sorting signals in its
C-terminal cytosolic domain (27) suggesting that multiple
forward targeting elements are required for correct
sorting to the micronemes. These signals may work
at distinct sites, however, since deletion of TgMIC2
does not cause retention of TgM2AP but instead results
in the misdirection of TgM2AP to the parasitophorous
vacuole. Such findings are not universal though because
deletion of the TgMIC6 propeptide has no effect on
sorting of TgMIC6 or its partner proteins (TgMIC1
and TgMIC4) to the micronemes (28). To determine the
type of protease involved in the proteolytic maturation
of microneme proteins, we tested a series of protease
inhibitors using the processing of nascent microneme
proteins as an indicator of activity. Whereas serine
or aspartyl protease inhibitors had no effect, cysteine
protease inhibitors delayed the processing of TgM2AP
and TgMIC3. Because cathepsin L inhibitor II was among
the most effective inhibitors, we hypothesized that
microneme proteins are processed by a cathepsin L-like
enzyme. Analysis of the Toxoplasma genome database
revealed that this parasite likely only has a single
cathepsin L-like gene, termed TgCPL. TgCPL features
a 30 kDa catalytic domain preceded by a proregion
that includes a transmembrane anchor, which is a unique
feature of apicomplexan cathepsin L proteases including
the falcipain family in Plasmodium (29). After purification,
recombinant proTgCPL undergoes autoactivation in vitro
under mildly acidic conditions. Although definitive
evidence is still forthcoming, several findings suggest
a link between TgCPL and microneme protein maturation.
First, the results of immunofluorescence and immunoelectron
microscopy experiments suggest that TgCPL occupies
a novel apical compartment intermediately positioned
between the trans-Golgi network and the mature micronemes.
Second, recombinant TgCPL can cleave recombinant proTgM2AP
at or very near the correct cleavage site. Third,
based on microarray screening of synthetic peptide
substrate libraries, recombinant TgCPL shows a preference
for leucine and structurally similar residues in the
P2 position, which is consistent with the cleavage
sites of TgM2AP, TgMIC3, TgMIC6, and TgAMA1, a microneme
protein recently shown to be necessary for Toxoplasma
invasion (30). Moreover, mutation of the TgM2AP P2
leucine residue to aspartic acid results in markedly
less efficient cleavage and partial use of an alternative
cleavage site 2 amino acids upstream of the normal
cut site. Additional studies to further test the role
of TgCPL in microneme protein maturation are underway.
A parallel may also exist in Sarcocystis muris since
this parasite expresses both a cathepsin L like enzyme
(SmTP1; (31)) and a microneme protein (16/17 kDa antigen;
(32)) that undergoes proteolytic maturation at a cleavage
site with a P2 leucine.
Figure2. Selective
proteolysis of Toxoplasma invasion proteins.
Microneme and rhoptry proteins are often multiply
processed both within the secretory pathway and on
the parasite surface whereas DG proteins are not subjected
to postranslational processing.
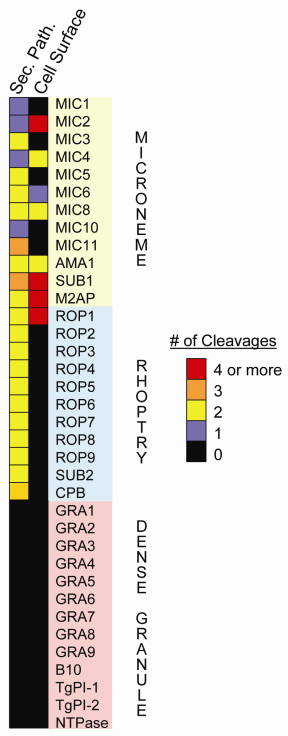
Mobilization of invasion proteins
While screening a small
library of cysteine protease inhibitors in an invasion
assay, we noted that two compounds substantially impaired
invasion while simultaneously disrupting microneme
protein release. These compounds LHVS and ZL3VS both
feature a vinyl sulfone warhead for electrophilic
attack of the active site cysteine of a thiolprotease.
LHVS and ZL3VS showed low micromolar dose-dependent
inhibition of parasite entry in two different invasion
assays, and had similar potency for blocking microneme
protein release. We focused on LHVS for subsequent
experiments because it was slightly more potent than
ZL3VS. Using fluorescent differential 2-dimensional
gel electrophoresis (2D-DIGE), we showed that LHVS
impaired the release of a variety of microneme proteins
but did not affect secretion of DG proteins or proteins
released from other internal sites. LHVS also disrupted
gliding motility since treated parasites fewer and
shorter trails compared to solvent or control compound
treated parasites. We initially reasoned that these
compounds may block gliding and invasion by preventing
the proteolytic shedding of microneme proteins from
the parasite surface. However, the recent evidence
that serine proteases of the rhomboid family are responsible
for microneme protein shedding (see below) is inconsistent
with this notion. Also, unlike the serine protease
inhibitor 3,4 DCI, LHVS treatment did not result in
the accumulation of microneme proteins such as TgMIC2
and TgM2AP on the parasite surface after stimulating
secretion with a calcium agonist. Based on these findings,
we conclude that a cysteine protease is required at
an earlier step, perhaps facilitating the mobilization
of microneme contents to the parasite surface. Interestingly,
a fluorescent derivative of LHVS (Bodipy-LHVS) covalently
labels a single 30 kDa band that immunoprecipitates
with TgCPL antibodies. Labeling was block by pretreatment
with LHVS, suggesting that the recognition is highly
specific. Moreover, bodypy-LHVS illuminates the same
apical compartment occupied by TgCPL. Whether TgCPL’s
role in the mobilization of microneme proteins is
related to its putative function as a maturase remains
under investigation.
Surface trimming
(primary processing)
After being discharged
from the micronemes, several invasion proteins are
subjected to proteolytic cleavages that do not affect
their association with the parasite surface. These
events are termed primary processing or “trimming”
because they occur before microneme proteins are proteolytically
liberated from the surface (see below). Amino or carboxy
terminal peptides of low structural complexity are
often the targets of trimming, which often occurs
at multiple sites in the same substrate. For example,
an amino-terminal peptide extending from the globular
A-domain of TgMIC2 is removed with with at least three
endoproteolytic cleavages by microneme protein protease
2 (MPP2) activity (33). This processing was recently
shown to activate the TgMIC2 A-domain for binding
to ICAM1, which the parasite uses in part to traverse
cell barriers to reach deep tissues where it replicates
(34). Therefore, disrupting MPP2 activity is predicted
to interfere with the pathogenesis of infection. MPP2
also cleaves TgMIC4 near its carboxy terminus and
it trims off the carboxy terminal “coiled”
domain from TgM2AP, along with another putative proteolytic
activity MPP3 (35). Although all of the MPP2 cleavage
sites for TgMIC2 and TgM2AP have been defined, this
information was not particularly revealing, apart
from suggesting that MPP2 prefers small to medium
sized uncharged amino acids in the P1-P4 sites. Whereas
MPP3 activity is resistant to all of the inhibitors
tested, MPP2 activity is blocked by the tripeptide
aldehyde compounds ALLN and ALLM and by the serine
protease inhibitors chymostatin and PMSF (35). Although
it was recently proposed (36) that surface trimming
is responsible for activating adhesive proteins for
tight binding to host receptors, little evidence exists
to support this idea. Critical to testing this hypothesis
is the identification and characterization of the
protease(s) responsible for MPP2 and MPP3 activities.
Intriguing new findings from collaborative studies
in between our labs (Carruthers and Kim) suggest a
potential breakthrough in this quest.
While investigating phenotypic changes stemming from
the targeted deletion of the TgSUB1 gene, we noted
from western blots that the trimming of TgMIC2, TgM2AP,
and TgMIC4 was markedly diminished in the TgSUB1 knockout
(KO) parasites compared to a control parasite line.
This microneme derived subtilase uses a glycosylphosphatidyl
inositol (GPI) anchor to transiently occupy the parasite
surface before being proteolytically shed into the
culture supernatant where it becomes a major component
of the excreted/secreted antigen (ESA) fraction (37).
To more widely examine changes in the processing of
microneme proteins, we performed 2D-DIGE on ESA fractions
collected from control parasites and TgSUB1KO. This
analysis vividly showed the near complete absence
of TgMIC2, TgM2AP, and TgMIC4 processing products,
with the corresponding accumulation of precursor species.
Since this pattern closely resembles that of ESAs
collected from ALLN treated parasites (35), we tentatively
conclude that TgSUB1 is MPP2. Although ALLN was initially
reported to be a selective inhibitor of calpains (calcium
dependent cysteine proteases), this compound also
has activity against some serine proteases including
the proteosome. Also, chymostatin and PMSF inhibition
of MPP2 is also consistent with it being TgSUB1. Since
subtilases are often activated by high calcium concentrations,
this may be how TgSUB1 is regulated upon reaching
the parasite surface and the extracellular environment.
Intriguingly, preliminary mouse infection experiments
suggest that TgSUB1KO parasites are moderately attenuated
in virulence, with some mice surviving a normally
lethal infectious dose. Further studies will be necessary
to determine whether this is due to an effect on cell
entry, tissue invasion, or both. Regardless, these
studies may have wider implications since orthologous
subtilases are expressed a variety of apicomplexans
including Neospora (38,39) and Plasmodium (40).
Surface shedding
(secondary processing)
In contrast to surface
antigens (SAGs) which continuously occupy the parasite
surface, invasion proteins derived from the microneme
are only transiently associated with the parasite
plasma membrane. Consequently, steady state levels
of microneme proteins on extracellular tachyzoites
are generally low. Microneme proteins are most readily
detected on the parasite surface during invasion,
where they can be seen accumulating on the extracellular
portion of the parasite as they translocate backwards
driven by the actin-myosin motor system. Our early
studies on TgMIC2 indicated that this protein was
shed into the culture supernatant as a smaller species
that was devoid of its carboxy-terminal cytosolic
domain and transmembrane anchor. This finding coupled
with the observation that TgMIC2 is not seen on intracellular
parasites suggested that microneme proteins are proteolytically
released from the parasite surface during invasion.
MPP1, the hypothetical protease responsible for shedding
is unaffected by a wide range of protease inhibitors
(33), suggesting it may be an unusual enzyme. This
notion was subsequently corroborated when Dominique
Soldati’s group demonstrated that TgMIC6 is
cleaved within its transmembrane anchor near the extracellular
interface. Although this suggested the existence of
an intramembranous protease, David Sibley’s
group simultaneously reported that mutation of two
lysine residues outside the transmembrane anchor abolished
shedding and disrupted invasion (41). To address this
apparent discrepancy, Zhou et al. (35) used mass spectroscopy
to determine that TgMIC2 is also cleaved intramembranously
at a site precisely corresponding to that of TgMIC6.
Consolidating these findings, it appears likely that
while cleavage occurs within the transmembrane anchor,
sequences outside of the anchor are required for protease
recognition or for supporting a favorable structural
configuration for cleavage.
Intramembrane proteolysis is a recently described
phenomenon performed by integral membrane proteases
that usually do not cleave until another protease
has processed the substrate at another site (42).
However, inhibition of MPP2 processing of TgMIC2 had
no effect on its shedding, implying that MPP1 does
not require a primary processing event. This clue
helped focus attention on the rhomboid family of intramembrane
serine proteases, which can cleave their substrates
without prior processing. Also, rhomboids typically
cleave near the extracellular portion of the transmembrane
anchor in a region populated by small, helix breaking
amino acids such as alanine and glycine (43), properties
that are consistent with the TgMIC2 and TgMIC6 cleavage
sites. Similar highly conserved putative cleavage
sites are seen a multitude of transmembrane microneme
proteins expressed by Neospora, Eimeria, and Plasmodium,
among other apicomplexans (44). Rhomboids are widely
present throughout the phylum (44). Heterologous expression
of fusion proteins with microneme transmembrane anchors
showed susceptibility to cleavage by human and drosophila
rhomboids (43). Analysis of the Toxoplasma genome
revealed the presence of six rhomboid-like genes (ROM1,
2, 3, 4, 5, and 6) (44-46). Since ROM6 is highly homologous
to a mitochondrial rhomboid involved in organellar
fusion, this was eliminated as a candidate for MPP1.
Also, ROM3 is not expressed in tachyzoites, reducing
the likelihood that it encodes MPP1. Localization
studies of the remaining candidates revealed that
ROM1 is expressed in the micronemes, ROM2 is in the
Golgi, ROM4 occupies the entire parasite surface,
while ROM5 is most abundant on the posterior surface
(45,46). Since MPP1 is constitutively active on the
parasite surface (33,47), ROM4 and ROM5 are currently
the best candidates. While ROM5 is the only ROM capable
of cleaving a full length fusion protein of TgMIC2
in a heterologous expression system (45), this protease
is not as well conserved among the Apicomplexa as
is ROM4 (46). Determining precisely which ROM is MPP1
will probably require conditional expression experiments.
Conclusions
Building on data from protease
inhibitor and cleavage site mapping, we and others
are using the effectively complete genome sequences
of Toxoplasma and other related parasites to begin
matching proteolytic events with the associated enzymes.
Emerging insight from these developments suggest that
proteases play distinct roles in the trafficking,
mobilization, and regulation of invasion proteins.
Cysteine proteases (TgCPB and TgCPL) along with the
subtilase TgSUB2 appear to participate in the proteolytic
maturation of microneme and rhoptry substrates during
the biogenesis of these organelles. Additionally,
TgSUB1 and integral membrane protease of the rhomboid
family function on the parasite surface where they
regulate proteins involved in cell entry and tissue
invasion. Future challenges not only include uncovering
a deeper understanding of the biological roles of
coccidian proteases but also the identification of
selective inhibitors designed to interfere with their
function for therapeutic gain.
Literature Cited
1. Waldner, C. L., Janzen,
E. D., and Ribble, C. S. (1998) J Am Vet Med Assoc
213, 685-690
2. Ruff, M. D. (1999) Vet Parasitol 84, 337-347
3. Montoya, J. G., and Liesenfeld, O. (2004) Lancet
363, 1965-1976
4. Dobrowolski, J. M., and Sibley, L. D. (1996) Cell
84, 933-939
5. Gaskins, E., Gilk, S., DeVore, N., Mann, T., Ward,
G., and Beckers, C. (2004) J Cell Biol 165, 383-393
6. Jewett, T. J., and Sibley, L. D. (2003) Mol Cell
11, 885-894
7. Carruthers, V. B., and Sibley, L. D. (1999) Mol
Microbiol 31, 421-428
8. Carruthers, V. B., and Sibley, L. D. (1997) Eur
J Cell Biol 73, 114-123
9. Hakansson, S., Charron, A. J., and Sibley, L. D.
(2001) Embo J 20, 3132-3144
10. Carruthers, V. B., Giddings, O. K., and Sibley,
L. D. (1999) Cell Microbiol 1, 225-235
11. Karsten, V., Qi, H., Beckers, C. J., Reddy, A.,
Dubremetz, J. F., Webster, P., and Joiner, K. A. (1998)
J Cell Biol 141, 1323-1333
12. Conseil, V., Soete, M., and Dubremetz, J. F. (1999)
Antimicrob Agents Chemother 43, 1358-1361
13. Adams, J. H., and Bushell, G. R. (1988) Int J
Parasitol 18, 683-685
14. Fuller, A. L., and McDougald, L. R. (1990) J Parasitol
76, 464-467
15. Que, X., Ngo, H., Lawton, J., Gray, M., Liu, Q.,
Engel, J., Brinen, L., Ghosh, P., Joiner, K. A., and
Reed, S. L. (2002) J Biol Chem 277, 25791-25797
16. Achbarou, A., Mercereau-Puijalon, O., Autheman,
J. M., Fortier, B., Camus, D., and Dubremetz, J. F.
(1991) Mol Biochem Parasitol 47, 223-233
17. Soldati, D., Lassen, A., Dubremetz, J. F., and
Boothroyd, J. C. (1998) Mol Biochem Parasitol 96,
37-48
18. Carey, K. L., Jongco, A. M., Kim, K., and Ward,
G. E. (2004) Eukaryot Cell 3, 1320-1330
19. Bradley, P. J., and Boothroyd, J. C. (2001) Int
J Parasitol 31, 1177-1186
20. Bradley, P. J., Li, N., and Boothroyd, J. C. (2004)
Mol Biochem Parasitol 137, 111-120
21. Bradley, P. J., Hsieh, C. L., and Boothroyd, J.
C. (2002) Mol Biochem Parasitol 125, 189-193
22. Kim, K., Soldati, D., and Boothroyd, J. C. (1993)
Science 262, 911-914
23. Shaw, M. K., Roos, D. S., and Tilney, L. G. (2002)
Microbes Infect 4, 119-132
24. Miller, S. A., Thathy, V., Ajioka, J. W., Blackman,
M. J., and Kim, K. (2003) Mol Microbiol 49, 883-894
25. Que, X., Wunderlich, A., Joiner, K. A., and Reed,
S. L. (2004) Infect Immun 72, 2915-2921
26. Cerede, O., Dubremetz, J. F., Bout, D., and Lebrun,
M. (2002) Embo J 21, 2526-2536
27. Di Cristina, M., Spaccapelo, R., Soldati, D.,
Bistoni, F., and Crisanti, A. (2000) Mol Cell Biol
20, 7332-7341
28. Reiss, M., Viebig, N., Brecht, S., Fourmaux, M.
N., Soete, M., Di Cristina, M., Dubremetz, J. F.,
and Soldati, D. (2001) J Cell Biol 152, 563-578
29. Rosenthal, P. J. (2004) Int J Parasitol 34, 1489-1499
30. Mital, J., Meissner, M., Soldati, D., and Ward,
G. E. (2005) Mol Biol Cell
31. Hansner, T., Freyer, B., Mehlhorn, H., and Ruger,
W. (1998) Parasitol Res 84, 578-582
32. Klein, H., Mehlhorn, H., and Ruger, W. (1996)
Parasitol Res 82, 468-474
33. Carruthers, V. B., Sherman, G. D., and Sibley,
L. D. (2000) J Biol Chem 275, 14346-14353
34. Barragan, A., Brossier, F., and Sibley, L. D.
(2005) Cell Microbiol 7, 561-568
35. Zhou, X. W., Blackman, M. J., Howell, S. A., and
Carruthers, V. B. (2004) Mol Cell Proteomics 3, 565-576
36. Carruthers, V. B., and Blackman, M. J. (2005)
Mol Microbiol 55, 1617-1630
37. Miller, S. A., Binder, E. M., Blackman, M. J.,
Carruthers, V. B., and Kim, K. (2001) J Biol Chem
276, 45341-45348
38. Louie, K., Nordhausen, R., Robinson, T. W., Barr,
B. C., and Conrad, P. A. (2002) J Parasitol 88, 1113-1119
39. Louie, K., and Conrad, P. A. (1999) Mol Biochem
Parasitol 103, 211-223
40. Blackman, M. J., Fujioka, H., Stafford, W. H.,
Sajid, M., Clough, B., Fleck, S. L., Aikawa, M., Grainger,
M., and Hackett, F. (1998) J Biol Chem 273, 23398-23409
41. Brossier, F., Jewett, T. J., Lovett, J. L., and
Sibley, L. D. (2003) J Biol Chem 278, 6229-6234
42. Brown, M. S., Ye, J., Rawson, R. B., and Goldstein,
J. L. (2000) Cell 100, 391-398
43. Urban, S., and Freeman, M. (2003) Mol Cell 11,
1425-1434
44. Dowse, T. J., and Soldati, D. (2005) Trends Parasitol
21, 254-258
45. Brossier, F., Jewett, T. J., Sibley, L. D., and
Urban, S. (2005) Proc Natl Acad Sci U S A 102, 4146-4151
46. Dowse, T. J., Pascall, J. C., Brown, K. D., and
Soldati, D. (2005) Int J Parasitol 35, 747-756
47. Opitz, C., Di Cristina, M., Reiss, M., Ruppert,
T., Crisanti, A., and Soldati, D. (2002) Embo J 21,
1577-1585